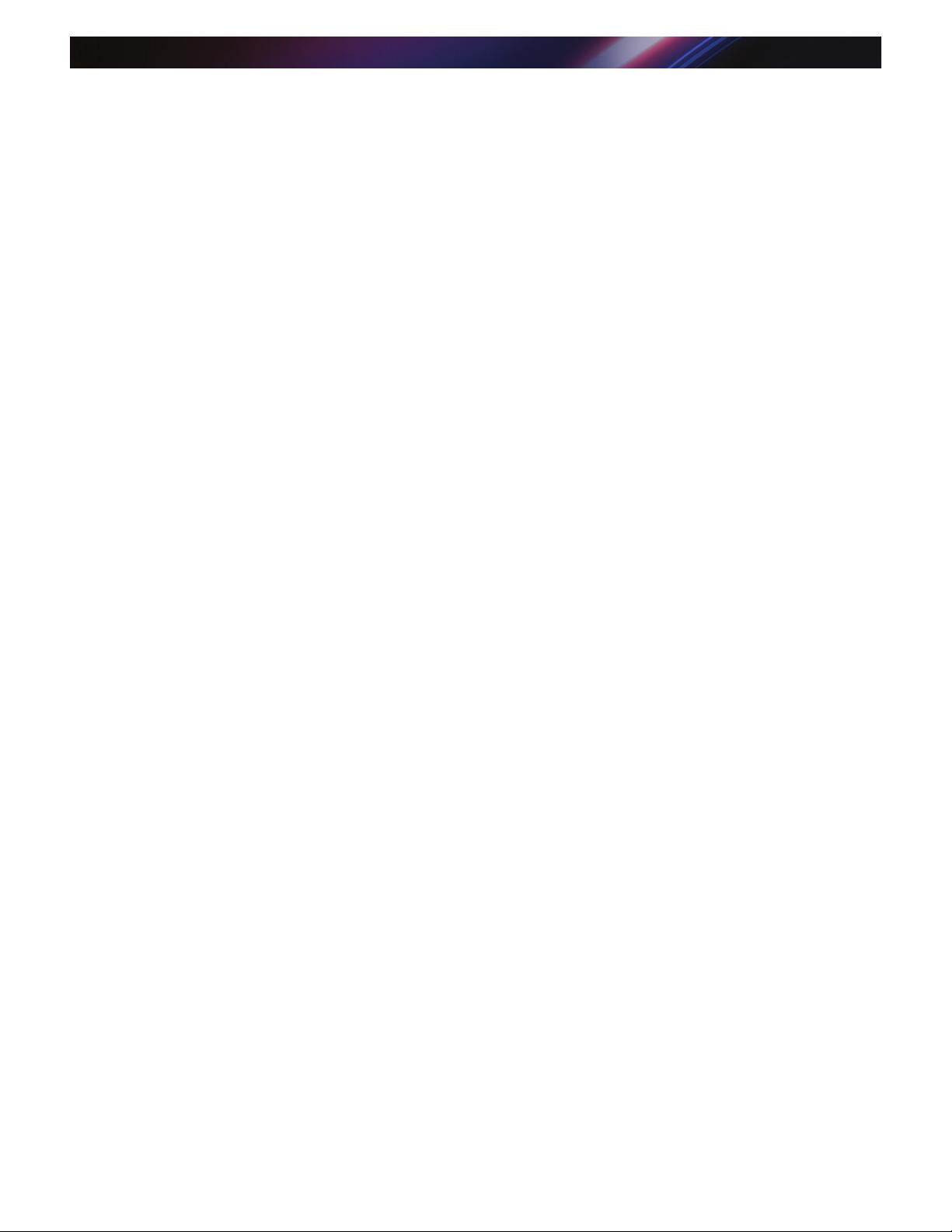
(MO, Beck Optronics Solutions, model 5003-000) with NA ¼
0.4 and with a working distance of 14.5 mm. Using a reflective
MO minimizes dispersion that would otherwise result in a
varying fringe spacing in the recorded spectral interferograms.
After the BS and the reflective MO, <1mWis incident on the
sample and tip. For these input powers, laser-induced tip- and/or
sample-heating effects were not observed. 80% of the incident
light is transmitted at the BS and serves as a reference field E
R
in
our measurements. For this, the power is adjusted by a variable
gray filter before it is reflected back to the BS by a mirror (M
R
)
that forms the reference arm of the Michelson interferometer.
The reference arm length is adjusted to be shorter than the sam-
ple arm by ∼100 μm in order to enable spectral interference
with a convenient fringe spacing and is then kept constant dur-
ing the measurement. To access the near-field region, a sharply
etched gold nanotaper is brought in close proximity to the
sample surface. Scattered light is collected in the backward di-
rection by the MO. The part that is transmitted through the BS is
overlapped with the reference field E
R
, and the resulting super-
position is collected for detection.
The near-field probe is a sharply etched single-crystalline
gold nanotip with an apex radius of curvature of ∼10 nm. The
tip is brought in close vicinity to the sample surface and the tip–
sample distance is modulated at a frequency f ≈ 26 kHz and
with an amplitude of 12 nm. The minimum tip–sample distance
is kept at 4 nm. Here, we set the light polarization to s, i.e., the
electric field vector is along the y axis such that it lies within the
sample plane and is perpendicular to the tip axis.
We employ two different detection methods. In the first case,
for quasimonochromatic measurements, the reference arm is
blocked and the signal backscattered from the focus region is
detected with an avalanche photodiode (APD, Hamamatsu,
model C5331-02). It is further processed by a lock-in amplifier
(Zurich Instruments HFLI), with the tip modulation frequency
as the reference signal. The signal demodulated at the funda-
mental tip modulation frequen cy as well as those demodulated
at its second, third, and fourth harmonics are obtained and
stored. We label these spectrally unresolved signals produced
by the lock-in amplifier with S
1f
, S
2f
, S
3f
, and S
4f
. In this case,
with the reference arm blocked, the signal detected by the APD
is constituted of light scattered out of the near field by the sharp
tip, E
NF
, and of the unwanted background field E
B
scattered
from the sample and from the tip shaft. Together, this results
in a voltage proportional to jE
NF
þ E
B
j
2
. Demodulating the
signal at higher-order multiples of the tip modulation frequency
makes the signal sensitive to the near-field contribution by re-
cording an interference pattern between E
NF
and E
B
.
39
In the second case, for spectrally resolved measurements,
the reference arm is unblocked and the output of the Michelson
interferometer is steered to the monochromator (Princeton
Instruments, IsoPlane-160) equipped with a fast line camera
(e2V AViiVA EM4 with 512 pixels). The spectra measured
now constitute a mixture of near field, background, and refer-
ence field:
SðλÞ ∝ jE
NF
þ E
B
þ E
R
j
2
: (1)
In front of the monochromator, the power detected from the
sample arm alone amounts to 10 μW, and the power detected
from the reference arm alone is 46 μ W. The reference arm
length is adjusted such that spectral interference fringes emerge
with a spacing of 3 to 5 nm (see the inset at the lower right in
Fig. 1). Equation (1) can be written as
SðλÞ ∝ jE
NF
þ E
B
j
2
þjE
R
j
2
þ 2Re½ðE
NF
þ E
B
Þ · E
R
· cosðk · ΔLÞ; (2)
where the wavevector k ¼ 2πλ
−1
and the path length difference
between sample and reference arm is ΔL. By separating the
modulated part of the measured spectra, the term RefðE
NF
þ
E
B
Þ · E
R
g is isolated and the complexity of Eq. (2) is consider-
ably reduced.
The remaining problem of suppressing E
B
with respect
to E
NF
is solved by the fast line camera behind the mono-
chromator.Thelinecamerahasamaximumreadoutrateof
210 kHz > 8 · f, and we typically record 60,000 consecutive
spectra with the maximum rate. In postprocessing, the recorded
values are considered separately, i.e., for each wavelength com-
ponent of the spectrum SðλÞ, the line camera records a time
series of 60,000 values. A Fourier series expansion is performed
around multiples of the tip modulation frequency. For each
pixel, the so-determ ined Fourier coefficients are quantities
analogous to the signals generated by the lock-in amplifier in
the monochromatic measurements. Assembling the respective
Fourier components for all camera pixels results in spectra
S
1f
ðλÞ to S
4f
ðλÞ, demodulated at the first to fourth harmonic
of the tip modulation frequency.
44
As we will show below, for
higher-order demodulation the background field E
B
is strongly
suppressed in comparison with the near-field E
NF
, such that
these spectra produce RefE
NF
E
R
g.
We investigate individual antimony sulfide (Sb
2
S
3
) nanopar-
ticles created on top of an Sb
2
S
3
film by EBL.
9
Our samp les are
annealed at 300°C to form crystalline thin films and nanostruc-
tures. This procedure shifts the bandgap from 2.24 to 1.73 eV,
and it reduces the above-gap absorption coefficient from 1 .8 ×
10
5
cm
−1
at 450 nm to 7.5 × 10
4
cm
−1
at 550 nm.
2
Here, we
study their optical properties at wavelengths between 700
and 900 nm, well below the bandgap. In this spectral region,
Sb
2
S
3
has relatively low losses (absorption <2 × 10
4
cm
−1
at
800 nm)
2
and a high index of refraction, with a positive real part
varying from 3.2 at 700 nm to 2.9 at 900 nm, which means that,
in our experiments, it can basically be considered as a lossless
dielectric.
Here, we cover a flat, 140-nm-thick film of crystalline Sb
2
S
3
with Sb
2
S
3
nanoparticles, aiming at a cylindrical structure that
should guide the incident light into the underlying thin film. The
nanoparticles are created by EBL
9
on top of the flat film in a
regular order, with a spacing of 2 μm [Fig. 2(a); more details
on the sample preparation can be found in the Supplemental
Materials]. The spacing is chosen sufficiently large to avoid
dipolar couplings between adjacent nanopar ticles such that
each nanodot may be considered as an isolated nanoparticle.
They are typi cally dome-shaped, with a round or slightly
elliptical cross section of ∼350 nm diameter and a height of
∼150 nm. Figure 2(a) shows a scanning electron microscope
(SEM) image of the nanoparticles. A higher resolution image
of an individual nanoparticle [Fig. 2(b)] reveals a slightly
elliptical shape and a somewhat rough surface. The center of
the particle seems to have collapsed to some extent after the
annealing proces ses following EBL. These features (ellipticity,
surface roughness, and indent ation) vary slightly from one
nanoparticle to the next.
Zhan et al.: Spatial and spectral mode mapping of a dielectric nanodot…
Advanced Photonics 046004-3 Jul∕Aug 2020
•
Vol. 2(4)