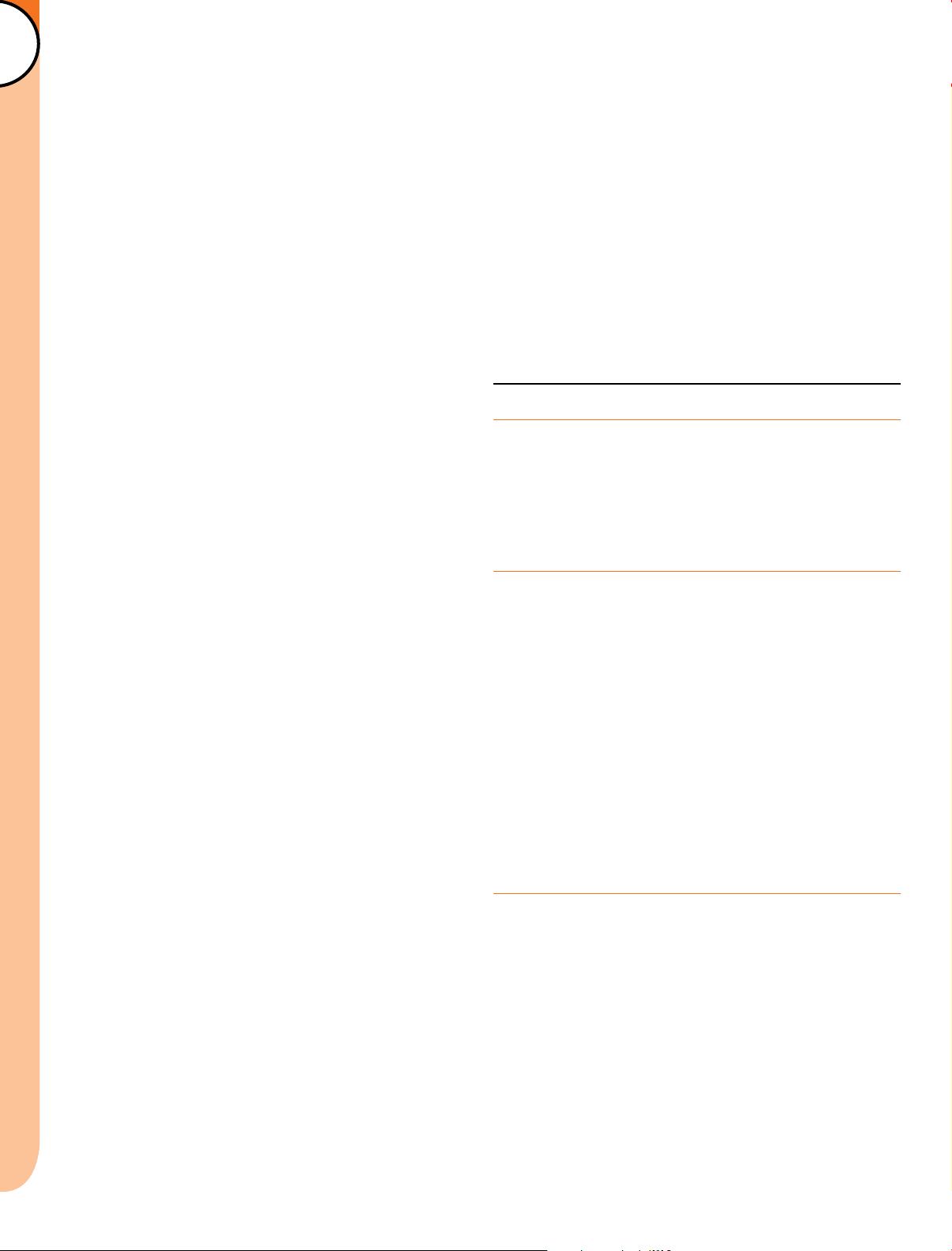
History of physiological optics in the twentieth century4
Introduction
Helmholtz (1821–1894), laid and cemented the foundations, and
ophthalmologists Donders (1818–1889), Landolt (1846–1926),
and Snellen (1834–1908) developed clinical applications. us,
a century ago, at the time of the beginning of the First World
War, a student had available comprehensive compendia containing
the available knowledge, specically Helmholtz’s Physiological
Optics in the new edition updated in particular by Gullstrand
(1862–1930), the Graefe-Saemisch Handbuch der Augenheilkunde
in its many volumes and several editions, followed in the next
couple of decades by important chapters in Vol XII of Bethe
etal., Handbuch der normalen und pathologischen Physiologie
(1932) and in Abderhalden’s Handbuch (1920), and in Vol 1
of Duke-Elder’s Textbook of Ophthalmology (1932). When the
author entered optometry school in 1940, the assigned textbook,
the second edition of Emsley’s Visual Optics (Emsley 1939),
contained a treatment of the subject that would rival current
accounts and in some respects exceed their scope. By that time,
too, quantum theory insofar as its characterization of the photon
was concerned had solid footing and quite soon thereafter gripped
the vision community when used to underpin our understanding
of the absolute visual threshold (Hecht etal. 1942). e vision
community has been well served by the authoritative treatment of
the subject by Yves LeGrand (1908–1986) in various versions of
his textbook (LeGrand 1949), a model of clarity.
Exhaustive literature surveys of vision science up to the
beginning of the twentieth century had been provided in the
encyclopedic scholarship displayed in the appendixes by A.
Koenig to the second edition of Helmholtz’s Physiological Optics
(almost 8000 references up to 1894) and F. Hofmann in his
Graefe-Saemisch Handbuch chapters (almost 1500 references on
spatial vision alone) and by A. Tschermak in the voluminous foot-
notes of his chapters in Bethe etal. Handbuch (Tschermak 1931).
As will be seen, developments in visual optics during the
second half of the twentieth century required an expanded view
beyond geometrical optics and the simple application of dirac-
tion theory in Airy’s disk, yet the foundations for it were well
in place. Abbe (1873) and Rayleigh (1896) in their treatment of
microscope resolution used a framework that contained, almost
explicitly, all the elements that were to become mainstream in the
Fourier theory of optics that has since become dominant. ough
it was more of academic than practical interest at the time, the
theory applicable to coherent light (and what is more challeng-
ing, partially coherent light) was put on the table by van Cittert
(1934), and so were the celebrated polynomials of Zernike (1934).
e upswing in the growth of optics, specically as it plays a
role in vision, in the middle of the twentieth century thus had
their origin elsewhere. Most prominently, it was the harnessing
of scientic and industrial resources in the conduct of the Second
World War that ended in 1945. e scientic community virtually
unanimously rallied behind the war eort, contributing insight and
inventiveness to a heady mixture that also included technological
innovation and industrial prowess. After the war, this continued
in university and some corporate laboratories, blossoming into a
research enterprise of unprecedented magnitude and productivity.
e sequence into cybernetics (Wiener 1948), information
theory (Shannon and Weaver 1949), and the linear systems
approach (Trimmer 1950) and control theory was seamless and
so was the progress toward labs equipped with oscilloscopes,
photomultipliers, digital computers, transistor devices, in time
followed by lasers, LCD, and deformable mirrors. For decades,
vision laboratories thrived on “war surplus” lenses, mirrors,
prisms, and lters. Light that was once generated by candles,
and had its intensity controlled by the inverse square law and
its duration by episcotister disks, was produced by lamps with
specic laments and then by high-pressure mercury arcs with
wavelength range restricted by narrowband interference lters
and intensity adjusted by neutral density wedges. To achieve
high retinal illuminance, the laments were imaged in the pupil
in Maxwellian view well before its optical subtleties had been
realized (Westheimer 1966).
1.3 STRUCTURAL OPTICS OF THE EYE
1.3.1 EYE DIMENSION AND AXIAL LENGTH
e ingenious method of measuring the eye’s axial length by
utilizing x-ray phosphenes (Goldmann and Hagen 1942) soon
gave way to sonography that in the form of corneal pachometry
(Molinari 1982) is in clinical use and now has become a reliable
means of evaluating the refractive needs associated with cataract
extraction (Hoer 1981).
1.3.2 CORNEA
Because it is the principal source of the eye’s refractive power
and because, unlike the other refractive surfaces, it is immedi-
ately accessible, the cornea has always attracted much attention.
Gullstrand in his appendix to the ird Edition of Helmholtz
went into much detail about the shape of the corneal surface and
the various means of measuring it. Keratoscopy and keratometry
formed a strong chapter in Abderhalden’s Handbuch. As contact
lenses became ubiquitous and their tting needed good information
of the corneal surface on which they rested and whose optical
properties they largely preempted, rapid and accurate measure-
ments of corneal curvature could be performed in the clinic
by cleverly designed electro-optical apparatuses, the subject of
continued attention and technical innovation (Fowler and Dave
1994). Polarization eects, which can be made visible, have been
ascribed to the cornea (Stanworth and Naylor 1950).
1.3.3 THE CRYSTALLINE LENS
e anterior and posterior surfaces of the crystalline lens are of
critical relevance in how the eye accommodates, that is, changes
focus under neural control by contraction of the ciliary muscle.
E.F. Fincham (1937a), in part using optical means, made the
major contribution to this topic in his monograph. It became
clear early in the optical modeling of the eye that the anterior
and posterior curvature of the lens could not fully account for its
total refractive power for a biologically realistic refractive index,
and giving the eye a solid interior core was not supported by
evidence. Hence the proposition that a remarkable proportion of
its total refractive power is provided by a refractive index gradient
had gained acceptance by the time Gullstrand wrote his 1911
appendix. More recent approaches show just how challenging a
topic this is (Campbell 1984, Pierscionek and Chan 1989).