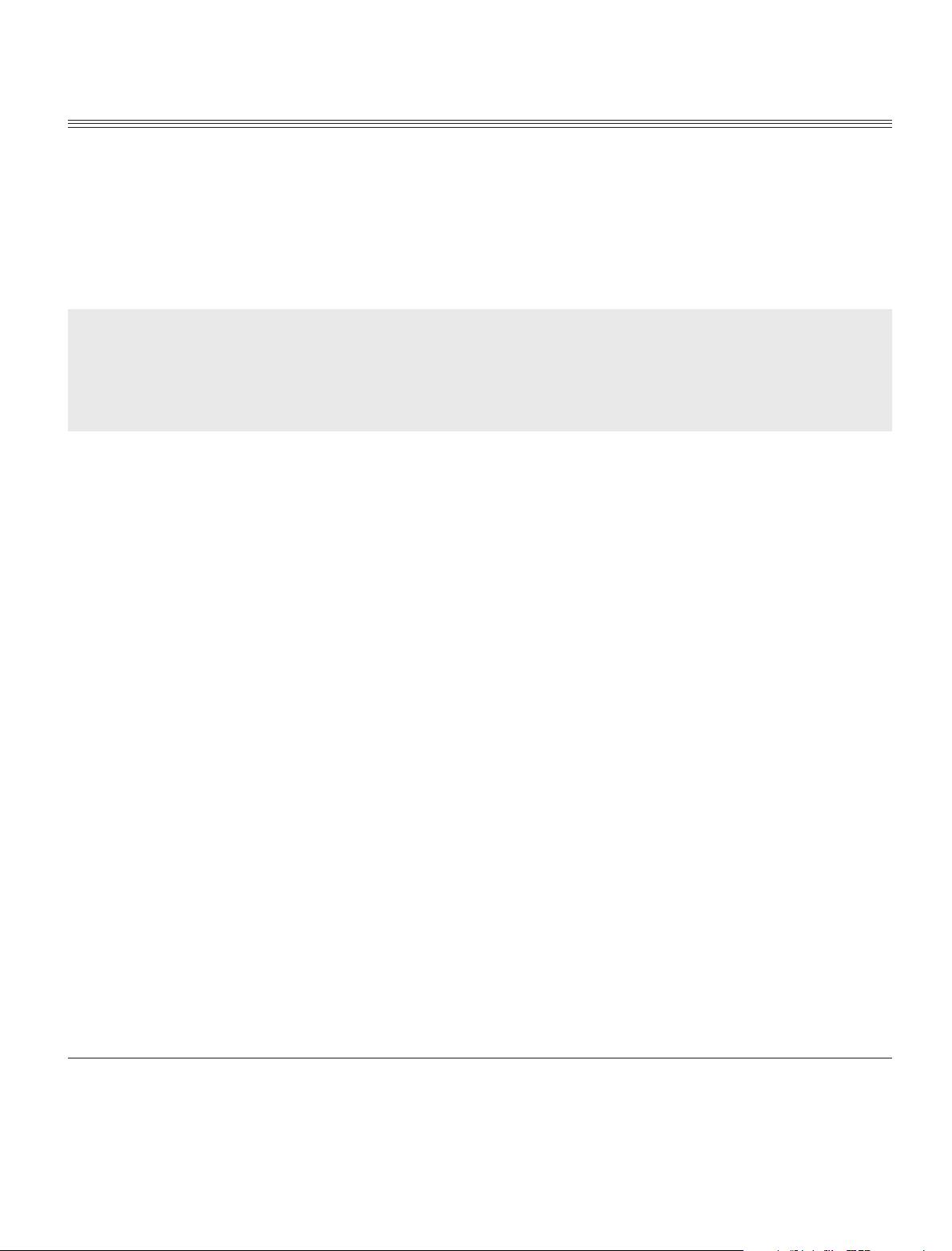
14 SEPTEMBER 2017 | VOL 549 | NATURE | 219
PERSPECTIVES
doi:10.1038/nature23884
The 4D nucleome project
Job Dekker
1
, Andrew S. Belmont
2
, Mitchell Guttman
3
, Victor O. Leshyk
4
, John T. Lis
5
, Stavros Lomvardas
6
, Leonid A. Mirny
7
,
Clodagh C. O’Shea
8
, Peter J. Park
9
, Bing Ren
10
, Joan C. Ritland Politz
11
, Jay Shendure
12
, Sheng Zhong
4
& the 4D Nucleome
Network*
T
he human genome contains over 20,000 genes and a larger number
of regulatory elements. Large-scale studies over the last decade
have catalogued these components of our genome and the cell
types in which they are active. The ENCODE, Roadmap Epigenome,
International Human Epigenome Consortium, EpiGeneSys (http://www.
epigenesys.eu/en/) and FANTOM projects
1–4
have annotated thousands
of genes and millions of candidate regulatory elements. However, our
understanding of the mechanisms by which these elements exert regula-
tory effects on specific target genes across distances of kilobases, and in
some cases megabases, remains incomplete.
The spatial folding of chromosomes and their organization in the
nucleus have profound effects on gene expression. For example, spatial
proximity is necessary for enhancers to modulate transcription of target
genes (for example, refs 5–7), and clustering of chromatin near the nuclear
lamina is correlated with gene silencing and replication timing
8,9
. In
addition, genome-wide association studies have identified large numbers
of disease-associated loci, and the majority of these loci are located in
distal, potentially regulatory, noncoding regions (for example, ref. 10).
In cancer cells, genomic rearrangements frequently occur and these
are at least in part guided by the three-dimensional organization of the
nucleus
11,12
. These data emphasize the importance of distal elements
for gene regulation and suggest an exciting opportunity to uncover the
fundamental mechanisms of disease through the mapping of long-range
chromatin interactions and three-dimensional genome organization.
Therefore, to determine how the genome operates, we need to understand
not only the linear encoding of information along chromosomes, but also
its three-dimensional organization and its dynamics across time, that is,
the ‘4D nucleome’. Concomitantly, we must pursue deeper knowledge
of the biophysical and molecular factors that determine genome
organization, and how this organization contributes to gene regulation
and other nuclear activities. Here we outline the goals and strategies of the
4D Nucleome (4DN) Network. This Network builds on other consortia
and efforts focusing on (epi-)genome analysis outlined above and adds
spatial and temporal dimensions to explore how the genome is organized
inside cells and how this relates to genome function.
The nucleus is not a homogeneous organelle, but consists of distinct
nuclear structures and non-chromatin bodies as well as defined chromo-
somal regions, such as centromeres, telomeres and insulator bodies, that
have been shown to cluster with each other and other genomic regions to
define distinct nuclear compartments
13,14
. Examples of nuclear structures
include the nuclear lamina and nuclear pores. Examples of nuclear com-
partments include the heterochromatic compartment, while examples of
nuclear bodies include nucleoli, nuclear speckles, paraspeckles, and Cajal
and PML (promyelocytic leukaemia) bodies. Chromosome conformation
capture (3C) approaches
15,16
have yielded additional insights by char-
acterizing genome-wide chromatin folding at kilobase resolution
6,17,18
.
These studies have shown that the genome is compartmentalized in active
and inactive spatial compartments at the scale of the nucleus, and that
within each compartment, folding of chromatin fibres brings together loci
and regulatory elements that are otherwise separated by large genomic
distances. CTCF, the cohesin complex and other DNA-binding proteins,
as well as RNAs, have roles in organizing chromatin domains and long-
range interactions between DNA loci
18–24
. These studies indicate that the
genome is intricately organized within the nucleus and that this organi-
zation has a critical role in gene regulation and activity.
During the past decade marked innovation in chromosome and
nuclear structure analysis has occurred. Genomic approaches for
mapping chromatin interactions, such as 3C, 4C (circular 3C, or 3C-on-
chip), 5C (3C-carbon copy), Hi-C, and chromatin-interaction analysis
by paired-end-tag sequencing (ChIA-PET)
16
, are yielding genome-wide
chromatin interaction maps at unprecedented resolution. Live-cell and
super-resolution microscopic approaches, combined with application of
new ways (for example, CRISPR–Cas9-based systems) to visualize loci
and sub-nuclear structures are beginning to provide detailed views of the
organization and dynamics of chromatin inside (living) cells
25–31
. There
has also been pronounced progress in analysing chromosome structural
data, producing structural models for chromosome folding
32,33
. However,
despite this progress, a comprehensive understanding of the 4D nucleome
is still lacking. This is partly due to the fact that different experimental cell
systems and approaches are used that together with the absence of shared
The 4D Nucleome Network aims to develop and apply approaches to map the structure and dynamics of the human and
mouse genomes in space and time with the goal of gaining deeper mechanistic insights into how the nucleus is organized
and functions. The project will develop and benchmark experimental and computational approaches for measuring
genome conformation and nuclear organization, and investigate how these contribute to gene regulation and other
genome functions. Validated experimental technologies will be combined with biophysical approaches to generate
quantitative models of spatial genome organization in different biological states, both in cell populations and in single cells.
1
Program in Systems Biology, Department of Biochemistry and Molecular Pharmacology, University of Massachusetts Medical School, Howard Hughes Medical Institute, Worcester, Massachusetts
01605, USA.
2
Department of Cell and Developmental Biology, University of Illinois, Urbana-Champaign, Illinois 61801, USA.
3
Division of Biology and Biological Engineering, California Institute of
Technology, Pasadena, California 91125, USA.
4
Department of Bioengineering, University of California San Diego, La Jolla, California 92093, USA.
5
Department of Molecular Biology and Genetics,
Cornell University, Ithaca, New York 14853, USA.
6
Department of Biochemistry and Molecular Biophysics, Mortimer B. Zuckerman Mind Brain and Behavior Institute, Columbia University,
New York, New York 10027, USA.
7
Institute for Medical Engineering and Science, and Department of Physics, Massachusetts Institute of Technology, Cambridge, Massachusetts 02139,
USA.
8
Molecular and Cell Biology Laboratory, Salk Institute for Biological Studies, La Jolla, California 92037, USA.
9
Department of Biomedical Informatics, Harvard Medical School, Boston,
Massachusetts 02115, USA.
10
Ludwig Institute for Cancer Research, Department of Cellular and Molecular Medicine, Institute of Genomic Medicine, Moores Cancer Center, University of California
San Diego, La Jolla California 92093, USA.
11
Basic Sciences, Fred Hutchinson Cancer Research Center, 1100 Fairview Avenue North, Seattle, Washington 98109, USA.
12
Department of Genome
Sciences, University of Washington, Howard Hughes Medical Institute, Seattle, Washington 98109, USA.
*A list of participants and their affiliations appears in the Supplementary Information.
© 2017 Macmillan Publishers Limited, part of Springer Nature. All rights reserved.